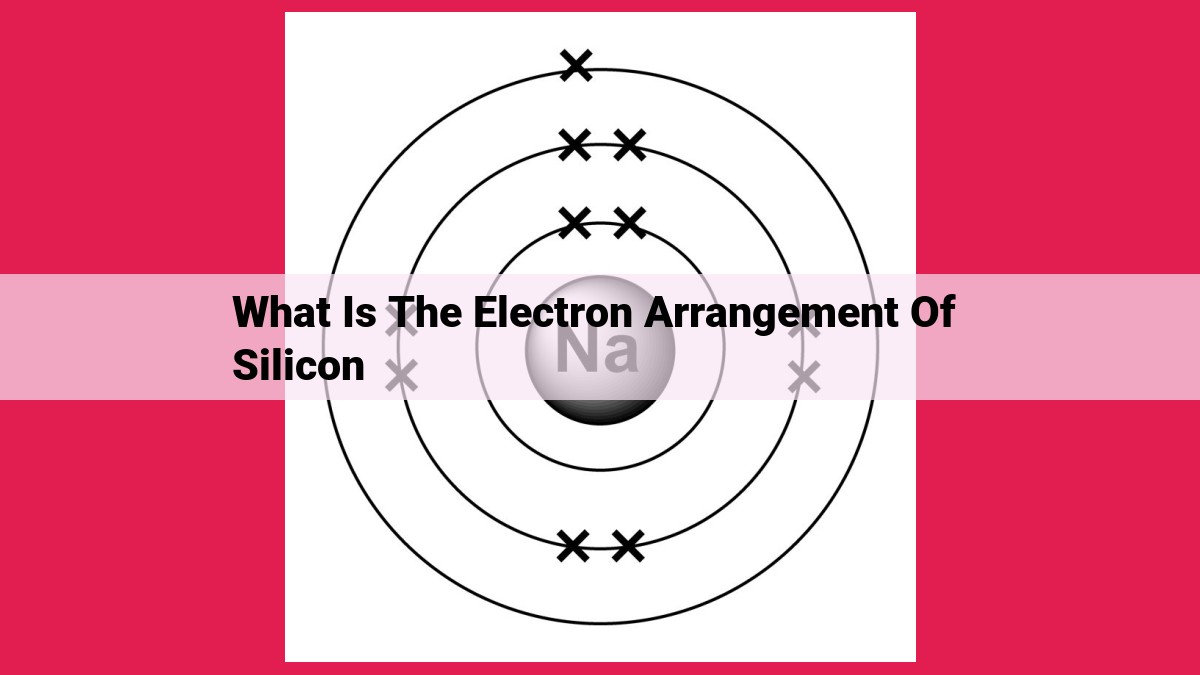
Silicon’s Versatile Chemical Properties: Unlocking Industrial And Electronic Applications
Silicon's unique electron arrangement plays a crucial role in its chemical properties. With four valence electrons and an electron configuration of [Ne]3s²3p², silicon readily forms covalent bonds. Its electron arrangement allows for tetrahedral hybridization, resulting in a tetrahedral molecular geometry in many of its compounds. This arrangement enables silicon to participate in diverse chemical bonding, making it a versatile element in various industrial and electronic applications.
Valence Electrons: The Foundation of Chemical Behavior
In the captivating world of chemistry, every element possesses a unique fingerprint that governs its behavior and interactions with others. This fingerprint is etched in the very arrangement of electrons that dance around its atomic nucleus. These electrons, known as valence electrons, hold the key to understanding an element's chemical personality.
Like the gears in a finely tuned machine, valence electrons determine how an element reacts, bonds with others, and participates in the symphony of chemical reactions. Take, for instance, the element sodium. It has a solitary valence electron, making it eager to shed this electron and become positively charged. This tendency explains why sodium is so reactive, readily forming bonds with elements that need an extra electron to complete their own electronic shells.
In contrast, the noble gases, such as helium and neon, possess a full complement of valence electrons. This makes them inert and reluctant to engage in chemical reactions, as their electron shells are already in a state of perfect harmony.
Thus, the number and arrangement of valence electrons dictate an element's chemical destiny. They shape its reactivity, determine its bonding preferences, and ultimately influence the myriad chemical transformations that occur in the natural world.
Unveiling the Electron Configuration: A Journey to Understanding Atomic Architecture
Atomic structure is the cornerstone of chemistry, and the electron configuration of an element holds the key to deciphering its chemical behavior. Electron configuration describes the specific arrangement of electrons within the concentric energy levels, or orbitals, surrounding the atom's nucleus. This atomic blueprint not only reveals the valence electrons that dictate reactivity, but also provides crucial insights into the element's atomic number and o
Understanding the Electron Configuration Code
Each electron within an atom occupies a unique orbital, with the lowest energy levels being filled first. The atomic number of an element, which uniquely identifies it, directly corresponds to the total number of electrons. This atomic number determines the sequence of filling electrons into orbitals, starting from the innermost energy level and transitioning outwards.
The valence electrons are those electrons residing in the outermost energy level. These electrons play a pivotal role in determining an element's chemical reactivity, as they are involved in the formation of chemical bonds with other atoms. Valence electrons are essential for understanding the bonding characteristics and chemical behavior of an element.
Electron Configuration and Valence Electrons: A Tale of Two
The electron configuration of an element reveals the number of valence electrons it possesses. For instance, hydrogen, with an atomic number of 1, has a single valence electron in its outermost energy level. In contrast, carbon, with an atomic number of 6, has four valence electrons.
Electron Configuration: A Guiding Light in Chemistry
The electron configuration of an element is not merely a theoretical abstraction. It serves as a practical tool in chemistry, aiding in the prediction and comprehension of chemical behavior. By understanding the electron configuration of an element, scientists can unravel its potential for forming chemical bonds, its reactivity with other elements, and its place in the periodic table.
Electron configuration stands as a fundamental pillar in understanding the chemical properties of elements. It establishes the foundation for comprehending valence electrons, ultimately providing a roadmap for unraveling the intricacies of chemical bonding and reactivity. As we continue to explore the realm of chemistry, the electron configuration of an element remains an invaluable guide, illuminating the path towards deciphering the atomic blueprint of the universe.
Lewis Structures: Unveiling the Blueprint of Molecular Connectivity
In the realm of chemistry, understanding the arrangement of electrons within atoms and molecules is crucial for deciphering their behavior and properties. Among the various tools employed to visualize electron distribution, Lewis structures stand out as indispensable aids in understanding atomic connectivity and chemical bonding.
What are Lewis Structures?
Lewis structures are diagrammatic representations that depict the arrangement of valence electrons in atoms or molecules. Valence electrons, the electrons residing in the outermost energy level of an atom, play a pivotal role in determining an element's chemical behavior. By depicting these valence electrons as dots or lines around the elemental symbols, Lewis structures provide insights into the electron arrangement and bonding patterns of atoms.
Significance of Lewis Structures
The significance of Lewis structures lies in their ability to elucidate the molecular geometry and chemical bonding within a molecule. By examining the arrangement of valence electrons, chemists can predict the shape of the molecule and the strength of the bonds between atoms. This understanding is essential for comprehending the chemical reactivity, physical properties, and behavior of substances.
For instance, the Lewis structure of carbon dioxide (CO2) reveals that the carbon atom is surrounded by two pairs of valence electrons, forming two double bonds with the oxygen atoms. This arrangement suggests a linear molecular geometry and strong covalent bonds between the carbon and oxygen atoms.
Drawing Lewis Structures
Drawing Lewis structures involves a systematic approach to distributing valence electrons around the atoms in a molecule. The following steps provide a general guideline:
- Determine the total number of valence electrons in the molecule.
- Connect the atoms with single bonds to represent the covalent bonds between them.
- Place the remaining valence electrons as pairs around the atoms to satisfy the octet rule.
- Adjust the bonding by converting lone pairs to double or triple bonds, as necessary, to achieve the most stable electron arrangement.
By following these steps, you can create Lewis structures that accurately represent the electron arrangement and bonding within a molecule, unlocking valuable insights into its chemical behavior and properties.
Electron Arrangement of Silicon: Unraveling the Atomic Puzzle
In the realm of chemistry, understanding the electron arrangement of elements is crucial to deciphering their chemical behavior. Among these elements, silicon stands out with its intriguing electron configuration that shapes its unique atomic properties.
Silicon, with its atomic number of 14, possesses four valence electrons in its outermost energy level. This specific arrangement of valence electrons, denoted as 2s²2p², sets the stage for silicon's distinctive chemical characteristics.
The Lewis structure of silicon further illuminates its electron arrangement, depicting the spatial arrangement of valence electrons and the formation of covalent bonds. Silicon's Lewis structure takes the form of :Si:, where the two dots represent the two valence electrons available for bonding. This electron arrangement reveals silicon's potential to form four covalent bonds with neighboring atoms.
The electron configuration of silicon also plays a pivotal role in determining its molecular geometry. The concept of molecular geometry refers to the three-dimensional arrangement of bonded atoms within a molecule. In the case of silicon, the availability of four valence electrons allows it to form tetrahedral molecular geometry, with four equivalent bonds extending in different directions from a central silicon atom.
Hybridization, the phenomenon of combining atomic orbitals to form hybrid orbitals, is a crucial factor influencing the molecular geometry of silicon compounds. Silicon undergoes sp³ hybridization, where one s orbital and three p orbitals combine to form four equivalent hybrid orbitals. The tetrahedral shape of these hybrid orbitals dictates the tetrahedral molecular geometry of silicon-containing molecules.
Understanding the electron arrangement of silicon is paramount for comprehending its chemical reactivity and bonding behavior. The presence of four valence electrons in silicon's outermost energy level enables it to readily form four covalent bonds, giving rise to its characteristic tetrahedral molecular geometry. These fundamental concepts lay the foundation for exploring the diverse chemical compounds and applications of silicon, a versatile element that plays a central role in modern technologies.
Molecular Geometry: Shaping the Three-Dimensional Structure
The world of chemistry revolves around electron arrangement, which determines how atoms interact and form the molecules that make up our world. Molecular geometry takes this concept a step further by describing the spatial arrangement of bonded atoms within a molecule. Imagine a molecule as a dance, with atoms as graceful dancers moving to the rhythm of electron configuration and hybridization.
Just as the number of valence electrons defines an element's chemical behavior, it also plays a crucial role in molecular geometry. This is because valence electrons are the ones that participate in chemical bonding, forming the bonds that hold atoms together. The distribution of valence electrons around the central atom determines its geometry.
Another key factor influencing molecular geometry is hybridization. This fascinating process involves the merging of atomic orbitals to form new hybrid orbitals with unique shapes and energy levels. Hybridization allows atoms to adopt specific geometries to minimize repulsion and maximize stability.
For instance, take methane (CH₄), a simple molecule with a tetrahedral geometry. Each carbon atom has four valence electrons and hybridizes its s and p orbitals to form four equivalent sp³ hybrid orbitals. These hybrid orbitals have a tetrahedral shape, resulting in the four C-H bonds pointing towards the corners of a tetrahedron.
Understanding molecular geometry is essential for comprehending the properties and reactivity of molecules. It provides valuable insights into their shape, polarity, and bonding characteristics. This knowledge empowers chemists to predict and design new molecules with desired properties, paving the way for advancements in fields like medicine, materials science, and energy research.
Hybridization: Merging Orbitals for Chemical Bonding
In the realm of chemistry, understanding the arrangement of electrons is paramount to unraveling the mysteries of atomic behavior and chemical bonding. Hybridization emerges as a pivotal concept in this enigmatic world, a process where atomic orbitals gracefully merge to forge hybrid orbitals. As we delve into this captivating tale, let us unveil how hybridization orchestrates molecular geometry and governs the strength of chemical bonds.
Hybridization, dear readers, is the art of harmoniously blending atomic orbitals, akin to a celestial dance where individual entities unite to form a cohesive whole. This enchanting process gives rise to hybrid orbitals, which, unlike their atomic counterparts, possess distinct shapes and energies. These hybrid orbitals, adorned with their newfound characteristics, play a pivotal role in shaping molecular geometry.
For instance, consider the ubiquitous carbon atom, a master of disguise with its chameleon-like ability to don various hybridization states. When carbon undergoes sp3 hybridization, its four valence electrons waltz together to form four equivalent hybrid orbitals, tetrahedrally arranged in space. This tetrahedral geometry becomes the foundation for carbon's remarkable bonding prowess, enabling it to form stable covalent bonds with a multitude of atoms.
Hybridization not only governs molecular geometry but also wields a profound influence over the strength of chemical bonds. Double bonds, boasting greater stability and shorter bond lengths, arise when hybrid orbitals overlap laterally. This intimate overlap results in the formation of a sigma bond and a pi bond, bestowing enhanced strength upon the molecular edifice.
In the captivating narrative of chemical bonding, hybridization emerges as an indispensable chapter. It unveils the intricate interplay between atomic orbitals and molecular geometry, elucidating the enigmatic dance of electrons that shapes the very essence of matter. By understanding the intricacies of hybridization, we gain invaluable insights into the fundamental forces that govern the chemical world around us.
Related Topics:
- Fire Truck Weight: Comprehensive Guide To Weight Regulations, Components, And Performance
- Copper: The Ultimate Conductor For Electrical Excellence
- Comprehensive Guide To Vehicles And The Automotive Industry | Riddle Included
- Understanding Mechanical Waves: Seismic And Sound Waves
- Unlock Educational Access: Comprehensive Strategies To Increase School Enrollment